Premium Only Content
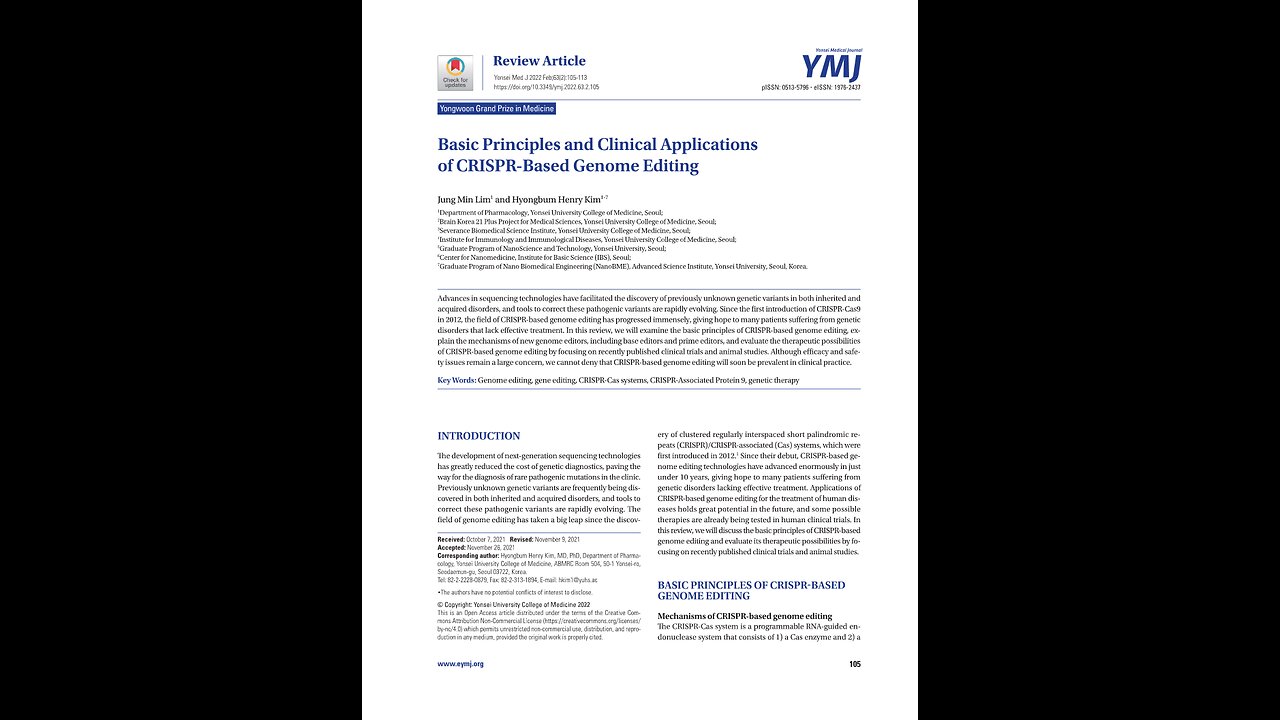
Basic Principles and Clinical Applications of CRISPER. Hyongbum Henry Kim A Puke(TM) Audiopaper.
Index of Other Science Articles:
https://rumble.com/v3t4yzj-index-of-science.-music-by-dan-vasc.html
Basic Principles and Clinical Applications of CRISPER-Based Genome Editing.
Review Article, Yongwoon Grand Prize in Medicine.
Corresponding author: Hyongbum Henry Kim, MD, PhD, E-mail: hkim1@yuhs.ac
Jung Min Lim, 2022
https://doi.org/10.3349/ymj.2022.63.2.105
Advances in sequencing technologies have facilitated the discovery of previously unknown genetic variants in both inherited and acquired disorders, and tools to correct these pathogenic variants are rapidly evolving. Since the first introduction of CRISPER-Cas9 in 2012, the field of CRISPER-based genome editing has progressed immensely, giving hope to many patients suffering from genetic disorders that lack effective treatment. In this review, we will examine the basic principles of CRISPER-based genome editing, explain the mechanisms of new genome editors, including base editors and prime editors, and evaluate the therapeutic possibilities of CRISPER-based genome editing by focusing on recently published clinical trials and animal studies. Although efficacy and safety issues remain a large concern, we cannot deny that CRISPER-based genome editing will soon be prevalent in clinical practice.
Key Words: Genome editing, gene editing, CRISPER-Cas systems, CRISPER-Associated Protein 9, genetic therapy
INTRODUCTION.
The development of next-generation sequencing technologies has greatly reduced the cost of genetic diagnostics, paving the way for the diagnosis of rare pathogenic mutations in the clinic. Previously unknown genetic variants are frequently being discovered in both inherited and acquired disorders, and tools to correct these pathogenic variants are rapidly evolving. The field of genome editing has taken a big leap since the discovery of clustered regularly interspaced short palindromic repeats (CRISPER)-CRISPER-associated (Cas) systems, which were first introduced in 2012. Since their debut, CRISPER-based genome editing technologies have advanced enormously in just under 10 years, giving hope to many patients suffering from genetic disorders lacking effective treatment. Applications of CRISPER-based genome editing for the treatment of human diseases holds great potential in the future, and some possible therapies are already being tested in human clinical trials. In this review, we will discuss the basic principles of CRISPER-based genome editing and evaluate its therapeutic possibilities by focusing on recently published clinical trials and animal studies.
BASIC PRINCIPLES OF CRISPER-BASED GENOME EDITING.
Mechanisms of CRISPER-based genome editing The CRISPER-Cas system is a programmable RNA-guided endonuclease system that consists of 1) a Cas enzyme and
2) a guide RNA (Figure 1A). Briefly, the Cas enzyme modifies a template by creating a double-strand break at a specific location, and guide RNA “guides” the Cas enzyme to the target sequence by simple Watson-Crick base pairing. Here, the single guide RNA (sgRNA) consists of two linked components, the CRISPERRNA (crRNA) and the trans-activating crRNA (tracrRNA). The target sequence (also called the protospacer) is recognized by the crRNA, which can easily be designed by an individual and thus makes the system programmable. The protospacer adjacent motif (PAM) is a short sequence directly after the protospacer that is recognized by the Cas enzyme and triggers its activity. The location of the double-strand break is related to the PAM, occurring three bases upstream of the PAM in the case of Cas9 (Figure 1A). The actual genome editing occurs in the process of repairing double-strand breaks created by the CRISPER-Cas system. Two main pathways are responsible for such repair: homology-directed repair and non-homologous end joining (NHEJ), with NHEJ predominant in mammalian cells (Figure 1A).
Figure 1. CRISPER-based genome editors.
A: Schematics of CRISPER-Cas9 and the results of CRISPER-Cas9-mediated genome editing.
B: Schematics of base editors and the results of base editing.
C: Schematics of prime editors and the results of prime editing. Cas9-induced cleavage sites are indicated with red vertical dashed lines. sgRNA, single guide RNA; PAM, protospacer adjacent motif; pegRNA, prime editing guide RNA; RT, reverse transcriptase.
The homology-directed repair strategy requires a donor template with homology to the context sequence, which integrates into a double-strand break site and results in precise genome editing.
Genome editing with homology-directed repair has been performed for quite a long time, but the efficiency of this process has been greatly enhanced by the CRISPER-Cas system. By using homology-directed repair, precise corrections, insertion of mutations, or insertion of genes of interest can all be done, although the co-delivery of donor templates is needed. Additionally, the efficiencies are generally low, and the process only occurs in dividing cells. On the other hand, NHEJ repairs a double-strand break in an almost stochastic manner, which results in small insertions or deletions at the double-strand break site. The final outcomes can vary depending on the repair, which frequently induces frameshift mutations resulting in depletion of target gene function. Although this strategy cannot induce precise mutations, it does not require a donor template, occurs in both dividing and non-dividing cells, and is generally more efficient.
Advances involving Cas9 variants.
Since the first introduction of the CRISPER-Cas9 system, numerous Cas9 variants have been discovered or engineered. First, Cas9 orthologs from various bacterial species were discovered. Cas9 from Streptococcus pyogenes (SpCas9) was the version initially used in genome editing, and smaller-sized variants, such as Cas9 from Streptococcus aureus (SaCas9), Neisseria meningitides (NmeCas9), or Campylobacter jejuni (CjeCas9), were found later. These variants recognize different PAM sequences and differ greatly in protein size, adding diversity to the toolkit. Second, different types of Cas enzymes have been discovered, and now several types of templates can be modified depending on the type of Cas enzyme. For example, Cas9 and Cas12 enzymes can cut DNA sequences, whereas Cas13 enzymes target RNA sequences. Third, high-fidelity variants, such as eSpCas9(1.1), SpCas9-HF1, HypaCas9, and evoCas9, were engineered to increase the specificity and clinical safety of genome editing outcomes. In general, increased fidelity is associated with reduced editing efficiency. Fourth, variants recognizing different PAMs were engineered to widen the targetable range of Cas9. Variants, such as xCas9, Cas9- NG, and SpRY, each recognize distinct PAM sequences, which enables the editing of previously non-targetable genomic sites.
Advances involving new genome editors.
With the development of Cas9 variants, new genome editors were engineered to enable precise genome editing in a different way. First, the fusion of a Cas9 nickase with a cytidine or adenosine deaminase resulted in the invention of base editors (Figure 1B), which enabled single nucleotide conversions in the genome. The guide RNA works the same way as before, guiding the Cas nickase to the target site. Then, the deaminase attached to the Cas9 nickase converts a single nucleotide within the editing window, which is normally between the third to eigtth nucleotide of the protospacer. Specifically, cytosine base editors use a cytidine deaminase to convert C to T, and adenine base editors use an adenosine deaminase to convert A to G.18 Because point mutations account for more than half of all known pathogenic genetic variants in humans, base editors hold large therapeutic potential for correcting disease-causing mutations in the clinic. Most importantly, Cas9 nickases rarely induce double-strand breaks and greatly decrease unnecessary NHEJ-mediated genomic alterations, which is critical for clinical applications. Most recently, researchers attached a reverse transcriptase onto a Cas9 nickase, resulting in the creation of prime editors (Figure 1C), which can generate almost any type of genome edit and therefore hold the greatest potential among systems developed to date. Here, the reverse transcriptase is paired with a prime editing guide RNA (pegRNA), which acts both as a guide RNA for the Cas9 nickase and an RNA template for the reverse transcriptase to deliver the desired edit into the genome. With prime editing, all types of point mutations, insertions, and deletions can be precisely inserted or corrected simply by designing the appropriate pegRNA sequence, greatly expanding the capacity of the genome editing toolkit. Prime editing also produces very low levels of unintended edits, compared to conventional genome editors, and thus would be much safer to use. Therefore, although there is still much to be developed in this area, these new genome editors point to a brighter future for the application of CRISPER-based genome editing.
Delivery of the CRISPER system.
Delivering the CRISPER system to a therapeutic target is critical for achieving efficient genome editing. Delivery methods can differ a great deal depending on the therapeutic approach, which can be divided into ex vivo and in vivo strategies. In an ex vivo strategy, genome editing takes place in isolated patient-derived cells, after which the engineered cells can be expanded and re-introduced into the patient. In this case, the CRISPER system can be delivered into the cells using plasmid vectors, ribonucleoprotein complexes, nanoparticles, or viral vectors. Ex vivo delivery has several advantages. First, the delivery occurs on a cellular level, where the efficiency is generally higher, and can be easily assessed before clinical application of the cells. Additionally, the CRISPER system is not introduced into the body, which decreases the possibility of unnecessary genomic changes in non-target tissues. Finally, patient-derived cells are used, so there is less concern about host immune responses. However, patient-derived cells can be difficult to isolate, culture, and expand, which greatly limits the range of treatable disorders. In addition, when these genome-edited cells are re-introduced into patients, often only a limited fraction of the cells functionally integrate into the relevant tissue. On the other hand, in vivo genome editing takes place inside human tissues and can be applied to a broader range of diseases.
In this case, the CRISPER system is mostly delivered by nanoparticles or viral vectors. However, this method is much more complicated than an ex vivo strategy because multiple physical barriers may exist and host immune responses can occur, decreasing the efficiency of CRISPER system delivery and resulting in lower rates of intended genome editing. Adeno-associated virus (AAV) has been widely used for in vivo genome editing due to its high transduction efficiency. The biggest challenge with using AAVs is that there is a size limit for the delivered gene of interest, which is approximately 4.7 kilobases, limiting the delivery of sequences encoding large proteins, such as SpCas9, base editors, and prime editors, together with sequences encoding the guide RNAs. Several strategies have been developed to solve this problem: the genome editor can be split into two parts and delivered separately, which can later be combined and become fully functional, using splice receptors or inteins, or smaller-sized Cas9 variants encoded by sequences that fall below the size limit, such as SaCas95 or CjCas9, can be used. However, recent studies have reported integrations of the AAV genome into double-strand break sites generated by Cas925 and immune responses against AAV capsid proteins after exposure, raising safety and efficacy issues; this problem must be addressed in future research.
Compared to viral vectors, non-viral delivery systems possess many advantages: their cargo size is more flexible, they elicit less of an immune response, and they can be modified to target specific organs. Many types of non-viral delivery vectors, including lipid nanoparticles, gold nanoparticles, and extracellular vesicles, are being explored for use in the delivery of the CRISPER system. Recently, lipid nanoparticles were used to deliver CRISPER-Cas9 for the treatment of a genetic disease in a human clinical trial, highlighting the importance of biomaterial science in clinical applications. There is still much to be understood in this emerging field, which we believe will pave the road to safe and efficient gene therapy in the near future.
CLINICAL APPLICATIONS OF CRISPER-BASED GENOME EDITING.
Ex vivo genome editing The first clinical trial on CRISPER-based ex vivo genome editing attempted to treat human immunodeficiency virus type 1 (HIV-1) infection. Disruption of the CCR5 gene, which encodes an important co-receptor for viral entry, was induced by nucleofection of ribonucleoprotein complexes targeting CCR5 into patient-derived hematopoietic stem and progenitor cells (HSPCs), which were subsequently transferred back to the patient. A 27-year-old male with HIV-1 infection and acute lymphoblastic leukemia received the treatment and showed successful transplantation and long-term engraftment of CRISPER edited HSPCs. CCR5 disruption efficiencies ranged from 5.2 to 8.3 percent in bone marrow cells over 19 months, which was not adequate to achieve the curative target. Recently, a clinical trial attempting to treat severe monogenetic diseases with CRISPER-based genome editing reported promising results. Sickle cell disease and beta-thalassemia represent distinct groups of inherited hemoglobinopathies caused by mutations in the hemoglobin beta-subunit (HBB) gene, which lead to mutant, reduced, or absent beta-globin proteins. In this study, rather than the pathogenic HBB gene itself, an enhancer of the BCL11A gene, which is a transcription factor repressing gamma-globin synthesis, was targeted to restore the production of fetal hemoglobin and compensate for the mutated HBB gene. Patient-derived HSPCs were edited with CRISPER-Cas9 with a sgRNA targeting the BCL11A enhancer to produce gene-edited HSPCs called CTX001. One patient with transfusion-dependent beta-thalassemia and one patient with sickle cell disease were infused with a single dose of CTX001 after myeloablation. High edited allele frequencies and levels of fetal hemoglobin were maintained, and both patients avoided disease-related transfusion events over 21.5 and 16.6 months. Although a few serious adverse events were present in both patients (neutropenic pneumonia and veno-occlusive liver disease with sinusoidal obstruction syndrome in Patient 1; sepsis with neutropenia, cholelithiasis, and abdominal pain in Patient 2), they resolved with appropriate treatment. These trials are ongoing, with additional preliminary results broadly consistent with the original findings. CRISPER-based ex vivo genome editing has also been applied to the treatment of refractory cancers. The basic concept here is to enhance the natural anti-tumor responses of cytotoxic T cells by the removal of immune checkpoint modulator genes via CRISPER-Cas9. Two clinical trials using this strategy were recently published, with mixed success. In the first report, researchers attempted to treat various advanced, refractory cancers, including multiple myeloma and liposarcoma, via CRISPER-Cas9 genome editing For this purpose, T cells were isolated from cancer patients and engineered with CRISPER-Cas9 to remove the endogenous T cell receptor (TCR) and immune checkpoint molecule programmed cell death protein 1 (PD-1). Specifically, deletion of the TCR alpha chain (TRAC) gene, TCR beta chain (TRBC) gene, and PDCD1 gene was induced by electroporation of ribonucleoprotein complexes into patient-derived T cells. The edited T cells, named “NYCE” (NY-ESO-1 transduced CRISPER 3X edited cells), were subsequently injected into the patients intravenously. A total of 3 patients received treatment and showed stable engraftment of engineered T cells, although editing frequencies of the target genes in peripheral blood mononuclear cells were relatively low at 5 to 10 percent. No significant off-target editing or serious adverse events were noted. Clinically, only one patient showed tumor regression limited to early stages of treatment, and all tumors eventually progressed, resulting in termination of the trial.
Another similar trial, reported shortly thereafter, attempted to treat refractory non-small cell lung cancer with CRISPER-engineered patient-derived T cells by targeting the PD-1 gene. Specifically, disruption of the second exon of the PD-1 gene was induced by electroporation of Cas9- and sgRNA-encoding plasmids into patient-derived T cells. A total of 12 patients were treated; they showed stable viability and expansion of edited T cells, although the median gene editing efficiency was quite low at 5.81 percent. No significant off-target editing or serious adverse events were present. Clinically, only 2 patients showed stable disease at 8 weeks, all patients eventually had disease progression, and 11 patients died of disease progression.
In vivo genome editing.
In the first report of CRISPER-based in vivo genome editing, this technology was applied to transthyretin amyloidosis, or ATTR amyloidosis, which results from the accumulation of misfolded transthyretin (TTR) protein in tissues. ATTR amyloidosis is a monogenic disease, and almost all TTR proteins are produced in the liver, which makes the condition an excellent target for CRISPER-based in vivo genome editing. NTLA-2001, a livertrophic lipid nanoparticle system containing Cas9 mRNA and sgRNA targeting the human TTR gene, was designed to reduce circulating TTR protein levels in humans. Six patients with TTR mutations and sensory polyneuropathy were treated with a single injection of NTLA-2001, which reduced serum TTR levels by 52 percent in the low-dose group and 87 percent in the high-dose group after 4 weeks, with minimal side effects. The study is currently ongoing; serial measurements will continue to confirm the long-term durability and safety of the treatment. Although the results have not been reported yet, many groups are attempting to use CRISPER-based in vivo genome editing to treat monogenic diseases, including Leber congenital amaurosis 10. Tables 1 and 2 list ongoing clinical trials using ex vivo and in vivo CRISPER-based genome editing. Furthermore, extensive research on animal models continues to provide promising targets for further applications, which will be discussed in the next section.
Animal studies in the laboratory.
Leber congenital amaurosis comprises a group of early-onset childhood retinal dystrophies, with each subtype caused by mutations in different genes. LCA type 10 is caused by mutations in the CEP290 gene. CRISPER-based genome editing has been applied to humanized LCA10 mouse models; wild-type CEP290 expression was effectively restored by subretinal injection of a single AAV encoding both SaCas9 and sgRNA.37 LCA type 2 is caused by mutations in the RPE65 gene. The rd12 mouse model of LCA2 was subjected to subretinal injection of two AAVs encoding 1) SpCas9 and 2) sgRNA and donor DNA, resulting in the recovery of retinal function.
Recently, the same mouse model was successfully treated with subretinal injection of adenine base editors using RNPs or intein-mediated split AAV vectors, and prime editors using trans-splicing split AAV vectors, showing promise in therapeutic genome editing with new genome editors. Retinitis pigmentosa, which refers to a heterogeneous group of inherited ocular diseases that result in progressive retinal degeneration, consists of 92 different phenotypes and is caused by mutations in over 200 genes. AAV mediated gene transfer to treat retinitis pigmentosa has already been approved as the first AAV gene therapy in history, but basic studies on CRISPER-based genome editing for this purpose only started in 2016. Since then, many CRISPER-based approaches, each targeting a different gene, have achieved success in animal models. Specifically, the Nrl gene was depleted via NHEJ or repressed via an approach called CRISPER interference, both instigated by AAV vector delivery of CRISPER components, and the Mertk gene was corrected with a novel method called homology-independent targeted integration. The Pde6b gene was corrected via homology-directed repair, and the Rho gene was depleted via NHEJ, both induced by in vivo electroporation of Cas9-encoding plasmids. Hereditary tyrosinemia type 1, a lethal genetic disorder caused by mutations in the fumarylace to acetate hydrolase gene, results in the accumulation of toxic metabolites that lead to severe liver damage. CRISPER-based genome editing was first used in humanized mouse models of HT1 in 2014, and resulted in correction of the pathogenic mutations and rescue of the lethal phenotype. Mutation-corrected hepatocytes, which display a growth advantage over mutated hepatocytes, can repopulate the liver even at a very low editing frequency. Following this initial work, Cas9 variants, base editors, and prime editors have successfully rescued the lethal HT1 phenotype in adult mouse models. Phenylketonuria is an autosomal recessive liver disease caused by mutations in the phenylalanine hydroxylase gene, which may cause mental retardation due to the neurotoxicity of metabolites. In adult mouse models, intravenous injection of AAVs encoding an intein-split cytosine base editor successfully restored blood phenylalanine levels and reversed the PKU-associated fur color. Later, the conventional homology-directed repair approach also successfully ameliorated symptoms with the help of chemical modifiers. Ornithine transcarbamylase (OTC) deficiency, an X-linked metabolic disorder characterized by hyperammonemia, is caused by mutations in the OTC gene. Using a dual AAV system containing:
1) SaCas9-encoding sequences and
2) sgRNA-encoding sequences and donor DNA, OTC mutations were corrected by homology-directed repair, resulting in increased survival in mouse models. Duchenne muscular dystrophy is an inherited X-linked disease caused by mutations in the dystrophin gene. CRISPER-based genome editing was first used to correct mutations and restore expression of dystrophin in mouse zygotes in 2014. After this initial work, many research groups reported successful CRISPER-Cas9-mediated restoration of dystrophin expression, in adult mouse, dog, and pig models of DMD. Adenine base editing also effectively reversed DMD pathology in mouse embryos and adult mouse models. Amyotrophic lateral sclerosis (ALS) is a neurodegenerative disorder in which the progressive death of motor neurons results in paralysis. Several causative genes have been identified as underlying hereditary ALS, and mutations in SOD1 are responsible for most cases of ALS type 1. Recently, intravenous injection of AAV encoding SaCas9 and SOD1-targeting sgRNA was shown to delay disease onset and improve motor functions in ALS mouse models. Glycogen storage disease Ia, also known as von Gierke disease, is caused by pathogenic mutations in the glucose-6-phosphatase alpha subunit (G6PC) gene that result in the accumulation of glycogen throughout the body. Recently, the highly prevalent G6PC p.R83C variant was subjected to in vivo CRISPER-based genome editing in mouse models using two AAVs, one encoding SaCas9 and the other encoding sgRNA, resulting in normalization of G6Pase activity, reductions in serum insulin levels, and long-term survival. Hutchinson-Gilford progeria syndrome is caused by mutations in the lamin A (LMNA) gene. Recently, the LMNA c1824 C T mutation, which is found in over 90 percent of patients with HGPS, was corrected in transgenic mouse models using AAVs encoding split versions of the adenine base editor, resulting in improvement of vascular pathology and extension of life span.
This report demonstrated the potential of new genome editors for directly correcting point mutations to treat genetic disorders.
FUTURE DIRECTIONS.
In the future, the application of base editors and prime editors in human trials will be very exciting to watch, because many genetic disorders are caused by point mutations, which can be corrected by new types of genome editors with very low levels of unintended genomic alterations. Basic researchers are striving to increase the editing efficiency of base editors and prime editors, and given that positive reports in animal studies have already been achieved, we expect a striking increase in the number of clinical trials involving CRISPER-based genome editing with these new tools. Additionally, another exciting possibility is on the horizon: treating common, non-Mendelian disorders, such as osteoarthritis, hypertension, inflammatory skin disorders, and Alzheimer’s disease, with CRISPER-based genome editing. These studies attempt to prevent the development or progression of multifactorial diseases by targeting genes with critical roles in disease pathophysiology. For instance, interleukin-1 beta and MMP13, which are among the main catabolic factors involved in osteoarthritis development, were ablated to prevent the progression of osteoarthritis in surgically induced mouse models. Angiotensinogen, a key player in the renin-angiotensin system that regulates blood pressure, was deleted to prevent the development of hypertension in spontaneous hypertensive rats. The NLRP3 inflammasome, which is responsible for the activation of inflammatory responses, was disrupted in order to alleviate common inflammatory skin disorders, such as psoriasis or atopic dermatitis, in mouse models. Finally, beta-secretase 1, which is required for the production of amyloid beta proteins, was targeted to suppress cognitive deficits in mouse models of Alzheimer’s disease. Although there is still very much to be developed, targeting just the right genes that can inhibit pathologic pathways might provide effective treatments for chronic disorders for which there are currently only a few treatment options and no definite cure. In conclusion, extensive research in the CRISPER-based genome editing field is bringing cures for human genetic diseases closer to being in our grasp. Although efficacy and safety issues remain a large concern, we cannot deny that CRISPER-based genome editing will soon be prevalent in clinical practice. We watch with great enthusiasm, as we delete the letters ‘im’ from ‘impossible’ with CRISPER-based genome editing to make things ‘possible’.
ACKNOWLEDGEMENTS.
74 REFERENCES.
-
2:00:10
Bare Knuckle Fighting Championship
3 days agoCountdown to BKFC on DAZN HOLLYWOOD & FREE LIVE FIGHTS!
49.5K3 -
2:53:01
Jewels Jones Live ®
1 day agoA MAGA-NIFICENT YEAR | A Political Rendezvous - Ep. 103
129K32 -
29:54
Michael Franzese
15 hours agoCan Trump accomplish everything he promised? Piers Morgan Article Breakdown
122K53 -
2:08:19
Tactical Advisor
19 hours agoThe Vault Room Podcast 006 | Farwell 2024 New Plans for 2025
190K11 -
34:12
inspirePlay
1 day ago $5.73 earned🏆 The Grid Championship 2024 – Cass Meyer vs. Kelly Rudney | Epic Battle for Long Drive Glory!
89.9K8 -
17:50
BlackDiamondGunsandGear
16 hours ago $3.11 earnedTeach Me How to Build an AR-15
63.9K6 -
9:11
Space Ice
1 day agoFatman - Greatest Santa Claus Fighting Hitmen Movie Of Mel Gibson's Career - Best Movie Ever
121K48 -
42:38
Brewzle
1 day agoI Spent Too Much Money Bourbon Hunting In Kentucky
82.2K13 -
1:15:30
World Nomac
1 day agoMY FIRST DAY BACK in Manila Philippines 🇵🇭
63.9K10 -
13:19
Dr David Jockers
1 day ago $11.93 earned5 Dangerous Food Ingredients That Drive Inflammation
82.5K17