Premium Only Content
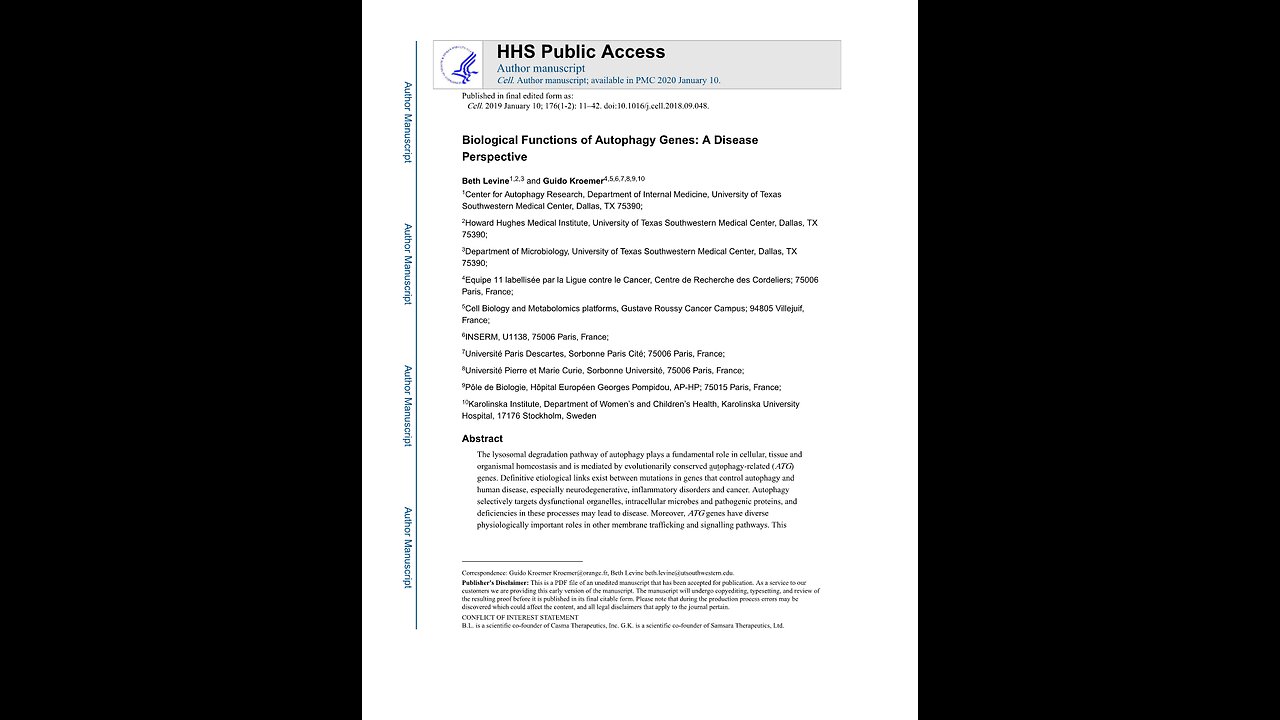
Biological Functions of Autophagy Genes. Beth Levine and Guido Kroemer. A Puke(TM) Audiopaper.
Biological Functions of Autophagy Genes: A Disease Perspective.
Beth Levine and Guido Kroemer.
Cell. 2019 January 10. Volume 176 (1 to 2). Pages 11 to 42.
doi:10.1016/j.cell.2018.09.048.
https://www.ncbi.nlm.nih.gov/pmc/articles/PMC6347410/
Index of other science articles:
https://rumble.com/v3t4yzj-index-of-science.-music-by-dan-vasc.html
Abstract.
The lysosomal degradation pathway of autophagy plays a fundamental role in cellular, tissue and organismal homeostasis and is mediated by evolutionarily conserved autophagy-related (ATG) genes. Definitive etiological links exist between mutations in genes that control autophagy and human disease, especially neurodegenerative, inflammatory disorders and cancer. Autophagy selectively targets dysfunctional organelles, intracellular microbes and pathogenic proteins, and deficiencies in these processes may lead to disease. Moreover, ATG genes have diverse physiologically important roles in other membrane trafficking and signalling pathways.
This review discusses the biological functions of autophagy genes from the perspective of understanding, and potentially reversing, the pathophysiology of human disease and aging.
Introduction.
A decade has elapsed since our review in 2008 in Cell on “Autophagy in the Pathogenesis of Disease”. During this period, more than 33,000 new articles related to autophagy were published, a Nobel prize was awarded for the discovery of the molecular mechanisms of autophagy to Levine and Klionsky in 2017, and to Mizushima in 2018. Considerable interest has also emerged in autophagy modulation as a potential target in clinical medicine.
The fundamental concepts discussed in our 2008 review remain unchanged. The lysosomal degradation pathway of macro autophagy, herein referred to as autophagy, plays a crucial role in cellular physiology, including adaptation to metabolic stress, the removal of dangerous cargo, for example, protein aggregates, damaged organelles, intracellular pathogens, the renovation during differentiation and development, and the prevention of genomic damage. Generally, these and other functions protect against numerous diseases, including infections, cancer, neurodegeneration, cardiovascular disorders, and aging. Under certain circumstances, autophagy may be detrimental either via its pro-survival effects, such as in cancer progression, or via possible cell death-promoting effects.
Over the past ten years, significant progress has been made in understanding the molecular mechanisms of autophagy, the regulation of autophagy, and the effects of autophagy on physiology and pathophysiology. New major conceptual advances underscore the plurality of functions of the autophagic core machinery in various membrane trafficking and signaling events and delineate the exquisite specificity with which autophagy targets selected cargo for degradation. These advances, together with discoveries in human genetics linking ATG gene mutations to specific diseases, provide a multidimensional perspective of mechanisms by which ATG gene-dependent pathways protect against mammalian disease.
Herein we review selected highlights of the past decade of research on the biological functions of autophagy genes, primarily from a perspective of understanding and treating human disease.
Autophagy and other Autophagy Gene-Dependent Pathways.
The original scientific definition of autophagy, from the Greek for “self-eating”, is the delivery of cytoplasmic cargo to the lysosome for degradation. There are at least three distinct forms of autophagy, chaperone mediated autophagy, micro autophagy and macro autophagy, which differ in terms of mode of cargo delivery to the lysosome. Macro autophagy is the major catabolic mechanism used by eukaryotic cells to maintain nutrient homeostasis and organelle quality control. It is mediated by a set of evolutionarily conserved genes, the autophagy-related (ATG) genes, originally discovered in yeast genetic screens.
With a few exceptions, all ATG genes are required for the efficient formation of sealed autophagosomes that proceed to fuse with lysosomes.
In higher eukaryotes, many ATG genes are functionally diversified to facilitate delivery of extracellular cargo to the lysosome, to promote the plasma membrane localization or extracellular release of intracellular cargo, and to coordinate intracellular communication with various cell signaling pathways, Figure 1. These other functions are not, sensu stricto, autophagy and accordingly, will be referred to as ATG gene-dependent pathways. There are broad implications of ATG gene functions in different membrane trafficking and signaling pathways for mammalian cell biology, physiology and disease.
Degradative Autophagy: The reason for the existence of Autophagy Genes.
The function of ATG genes as originally discovered is to orchestrate and mediate the formation of double-membraned structures that deliver intracytoplasmic contents to the lysosome for degradation. This process is conserved in all eukaryotic organisms, occurs at basal levels in nearly all cell types, and is increased by diverse intracellular and extracellular cues. It is essential for cellular homeostasis, cellular protein and organelle quality control, and organismal adaptation to environmental stress. These principles are firmly supported by nearly two decades of studies involving genetic ablation of the autophagy machinery in diverse eukaryotic species.
This lysosomal degradation pathway is usually described as involving a set of around 16 to 20 core conserved ATG genes. The ATG proteins encoded by these genes are traditionally classified into distinct biochemical and functional groups that act at specific stages of autophagosome initiation or formation. In this scheme, see other recent reviews for details, the ULK1 serine threonine kinase complex, involving ULK1, FIP200, ATG13 and ATG101, plays a major role in autophagy initiation, phosphorylating multiple downstream factors. Two distinct Beclin 1, class 83 phosphatidylinositol 3-kinase (PI3KC3) complexes generate phosphatidylinositol 3-phosphate (PI3P) to act in auto phagosome nucleation, or endolysosomal and auto phago lysosomal maturation. Vesicles containing ATG9A, the only transmembrane core ATG protein, supply membrane to auto phagosomes.
WIPI, WD repeat domain phosphoinositide-interacting, proteins and their binding partners, ATG2A or ATG2B, function in early stages of membrane elongation at the site of PI3P generation.
Autophagosome membrane expansion and completion involves two ubiquitin-like protein conjugation systems: the Ub-like ATG12 conjugates with ATG5 and ATL16L1 and the Ub-like LC3 subfamily, ATG8 in yeast, conjugates with membrane-resident phosphatidylethanoloamine, PE.
Unlike in yeast, the ubiquitin-like protein conjugation systems are not essential for auto phagosomal membrane completion in mammalian cells, although they determine the efficiency of the process.
This classification of the ATG proteins has provided a useful framework for studying and understanding autophagy. However, its apparent simplicity is at variance with extensive data indicating a highly complex level of interconnectivity among the ATG proteins and newly described functions of ATG proteins at different stages of autophagy.
Based on unbiased proteomic analyses, most ATG proteins interact with other ATG proteins that reside outside of their “classic” functional complex. Experimentally, some of these interactions are known to be important for autophagosome formation. For example, FIP200, a member of the ULK1 kinase complex, interacts with ATG 16L1 to properly target it to the isolation membrane, also known as the phagophore, of the nascent autophagosome. ATG14, a component of the autophagy-specific PI3KC3–C1 complex, also functions in SNARE-driven membrane fusion.
Similarly, Atg13, a component of the yeast Atg1, mammalian ULK1 kinase complex, interacts with Atg9 to recruit Atg9 vesicles to the pre-autophagosomal structure. The broader interconnectivity and functional multiplicity of core autophagy proteins in autophagosomal biogenesis requires further elucidation. Moreover, as indicated by a recent conditional genetic interactions study using diverse yeast omics datasets, new systems biology approaches will likely identify additional genes required for autophagy, especially those that may function in a stimulus-dependent, cell type-dependent or species-specific manner.
The core ATG proteins, conserved from yeast to humans, are necessary but not sufficient for degradative autophagy. The degradation of autophagosomal cargo cannot proceed without successful fusion to an available and functional lysosome. Research in the past decade has unmasked some of the key factors required for lysosomal biogenesis, autophagolysosomal fusion, lysosomal function during autophagy and autophagic lysosome reformation.
Adenoviral-mediated gene delivery of TFEB, a master transcriptional regulator of lysosomal biogenesis, improves outcomes in various rodent disease models, including Parkinson’s disease, lysosomal storage disorders, tauopathies, alpha 1-antitrypsin deficiency, and hepatic hyper-ammonemia.
Auto phago lysosomal fusion requires changes in lysosomal pH, certain cytoskeleton motor proteins (dynein), tethering factors, the HOPS complex, the Rab GTPase, RAB7, SNARE proteins, the Q-SNARE, syntaxin 17 on autophagosomes which interacts with R-SNARE proteins, SNAP29 and VAMP8 on endosomes, lysosomes, phospholipids, and members of the LC3 Gabarap family that are bridged to tethering factors or SNARES by adaptor proteins.
Screens in C elegans identified novel metazoan-specific genes required for fusion steps in degradative autophagy. One example relevant to human disease is EPG5, which encodes a RAB7 effector. Autosomal recessive mutation of EPG5 results in Vici syndrome, a neurodevelopmental and multisystem disorders, see Table 1. Mutations in genes that regulate lysosomal acidification such as ATP6AP2 and presenilin 1 are associated with X-linked Parkinsonism and Alzheimer’s disease, see Table 1. Thus, we must consider regulators of lysosomal biogenesis, the fusion machinery, and determinants of lysosomal function in our efforts to decipher how deficient autophagy leads to disease and how autophagy can be regulated to prevent or treat disease.
Beyond Self-Eating: Autophagy Genes Function in Phagocytosis.
Several core ATG genes function in a process that shares some similarities with autophagy but involves digestion of unwanted extracellular, rather than intracellular, material.
During this process, termed LC3-associated phagocytosis (LAP), single-membraned macroendocytic vacuoles, macropinosomes, phagosomes and entotic vacuoles, engulf extracellular cargo (such as bacteria, dead cells or live cells), become decorated by lipidated LC3, and are directed to the lysosome for degradation. LAP is distinguished from autophagy by four main features:
(1) The origin of the vacuolar contents, extracellular versus intracellular,
(2) The requirement of cargo engagement of an extracellular receptor for activation,
(3) The type of membrane that fuses with the lysosome, single membrane versus double membrane, and
(4) The utilization of a subset versus all of the core ATG proteins.
LAP requires NADPH-oxidase (NOX2) to generate reactive oxygen species (ROS), certain components of the Beclin 1, VPS34 complexes, PI3P generation, LC3-conjugation to the single membrane of the phagosome, and all components of the LC3 conjugation machinery.
However, it does not require other core ATG proteins, such as components of the ULK1 complex or the autophagy-specific Beclin 1, VPS34 complex component, ATG14. Somewhat enigmatically, LAP requires Rubicon, an inhibitory component of the autophagy-specific Beclin 1, VPS34 complex. The precise effects of LC3 decoration of phagosomes on their fusion with lysosomes and on lysosomal function are unknown. The presence of LC3 on phagosomes may enhance efficiency of phagolysosomal maturation, perhaps through a mechanism similar to that of LC3, GABARAP family members in autophagolysosomal maturation.
LAP was originally described in murine macrophages during phagocytosis of particles that engage Toll-like receptors (TLRs) and is involved in type I interferon (IFN) secretion in response to DNA immune complexes and other TLR9 ligands. Physiologically important functions of LAP have been identified by comparing phenotypes of mice with myeloid-specific deletion of LAP-specific genes, for example Rubicon or NOX2, and autophagy-specific ATG genes, for example FIP200 or Atg14.
LAP is required for degradation of photoreceptor outer segments by retinal pigment epithelium (RPE), a process necessary for intact vision. LAP is induced by the fungus, A fumigatus, and the intracellular bacterium, L monocytogenes, and enhances host defense against these pathogens. Mice lacking several components of the LAP pathway develop an autoimmune systemic lupus erythematosus (SLE)-like disease, perhaps due to a defect in the clearance of dying cells that triggers enhanced pro inflammatory signaling and autoantibody production.
Given the crucial roles of receptor-activated phagocytosis in human physiology, it is likely that LAP, like classical autophagy, will emerge as an important pathway in human disease. While the two pathways utilize overlapping genetic machinery, a critical distinction renders them antagonistic. Specifically, Rubicon is required for LAP but suppresses autophagy, and recent studies confirm a mutually inhibitory relationship between LAP and autophagy in photoreceptor degradation in RPE cells. It is not clear why these two pathways are counter-regulated, possibly, cells may need to shut off the alternative pathway during stress to avoid competition for overlapping resources. At a mechanistic level, it is uncertain how Rubicon functions to promote Beclin 1, VPS34 kinase activity at the phagosome, but inhibit it at other organellar sites.
Interestingly, the WD repeat-containing C-terminal domain of ATG16L1 is essential for LC3 recruitment to endolysosomal membranes in LAP, but dispensable for canonical autophagy, illustrating another difference in the molecular roles of an ATG protein in autophagy and LAP.
The genetic overlap and mutual antagonism of LAP and autophagy have practical implications for autophagy-targeted therapies. Theoretically, specificity in autophagy induction might be enhanced by activating the ULK1 complex rather than downstream shared nodes in autophagy and LAP (although the ULK1 kinase complex may have substrates outside of autophagy). The appeal of targeting Rubicon, a negative regulator of autophagy whose knockout in mice has beneficial effects, for example improved high-fat diet-induced hepatic steatosis and increased cardiac protection during lipopolysaccharide-induced sepsis, may be tempered by potential adverse effects of LAP inhibition, such as increased susceptibility to fungal diseases and autoimmunity. Furthermore, treatments that target shared ATG proteins may result in unpredictable effects on each pathway, assuming these proteins are rate-limiting and the two pathways compete for access to these shared core ATG proteins.
The identification of LAP as a lysosomal degradation pathway that utilizes certain core ATG genes requires us to adopt a wider interpretative lens for studies of mice with deletions of these genes. Does deficient LAP versus deficient autophagy contribute to pathological phenotypes in mice with whole body or tissue-specific deficiency of genes such as beclin 1, ATG5, ATG7, or ATG16L1? To what extent does increased autophagy versus decreased LAP contribute to Rubicon knockout phenotypes? Do the GWAS associations between polymorphisms in some of these genes and diseases that involve disordered immune regulation, such as asthma, SLE, and inflammatory bowel disease, see Table 1, suggest a role for altered LAP in their pathogenesis? The observation that a deficiency of LAP-associated, but not of non-LAP-associated, ATG genes results in a SLE-like syndrome in mice, underscores the importance of this question. Specific molecular markers to distinguish LAP from autophagy in both animal models and human disease are needed.
Beyond Lysosomal Degradation: Autophagy Genes Function in Secretion and Exocytosis.
ATG genes are used not only for targeting intracellular cargo to the lysosome for degradation, but also for pathways that involve the targeting of intracellular cargo to either the plasma membrane or extracellular environment, Figure 1. Generally, these pathways have been grouped under the umbrella term “secretory autophagy”, however, as the “phagy” part is missing from the process, we prefer the more linguistically precise term of ATG gene-dependent secretion. There are many different types of ATG gene-dependent secretion, reviewed elsewhere from a cell biology perspective, but the mechanisms governing most of these processes are not well understood. Here, we focus on these pathways as they may relate to mammalian physiology and disease.
Unconventional secretion involves the extracellular release of proteins that lack amino-terminal signal peptide leader sequences and bypass “conventional” transit through the endoplasmic-reticulum, ER, Golgi apparatus to reach the plasma membrane.
A role for ATG proteins in this process was first discovered in yeast secretion of the acyl-CoA-binding protein, Acb1. In mammalian cells, unconventional secretion of leaderless proteins, such as the pro-inflammatory cytokines processed by the inflammasome, IL-1 beta and IL-18, also require the autophagy protein, ATG5. The precise mechanisms underlying ATG gene-dependent unconventional secretion remain unclear. It is not certain whether targets are captured in an autophagosomal lumen and, or the intermembrane space between the double membrane of the autophagosome, nor is it certain how targets are delivered to and released from the plasma membrane. Autophagosome-like vesicles containing IL-1 beta bypass syntaxin 17-dependent fusion with lysosomes and instead use specific SNAREs and syntaxins involved in vesicle fusion with the plasma membrane for cargo secretion.
A function of ATG genes in secretion of pro-inflammatory mediators, and more broadly, other leaderless proteins, could have vast importance for inflammatory disorders and a wide range of other diseases. However, it is currently difficult to assess the physiological importance of ATG gene-dependent secretion of IL-1 beta and IL-18 in vivo, as macrophage, or hematopoietic cell specific deletion of Atg5, Atg16l1, and Atg7 in mice is associated with increased, rather than decreased, levels of IL-1 beta and IL-18 production.
These findings may reflect basal functions of ATG genes in the negative control of inflammasome activation, whereas the ATG gene-dependent secretion of pro-inflammatory mediators may be unmasked during certain stress conditions, such as inflammasome activation triggered by lysosomal membrane damage. The possibility of an autophagy-dependent secretome in vivo warrants further investigation and may lead to the identification of proteomic signatures of autophagy activation as clinically useful serum biomarkers. Theoretically, autophagy-inducing therapies might lead to untoward effects via the unconventional secretion of pro-inflammatory mediators or other pathogenic proteins.
Perhaps the best-established link between ATG gene-dependent secretion and mammalian physiology and disease relates to the exocytosis of secretory granules and lysosomes. Notably, human genome wide association studies (GWAS) that revealed a polymorphism in a core ATG gene, ATG 16 L1 T300A, as a major risk allele for Crohn’s disease spurred the discovery of a fundamental role for the ATG protein conjugation machinery in secretory granule exocytosis.
In mice, the hypomorphic expression of Atg 16 L1, referred to as the Atg 16 L1 T300A knock-in mutation, Paneth cell-specific deletion of Atg16L1, Atg5, or Atg7, or the whole-body deletion of Atg4b results in abnormal granule morphology and a defect in granule exocytosis and lysozyme secretion by Paneth cells. Paneth cells are a specialized ileal epithelial cell type that controls the intestinal microbiota by secreting lysozyme and antimicrobial peptides. Similar defects in Paneth cell morphology are observed in patients with, but not those without, the ATG 16 L1 T300A Crohn’s disease risk allele.
The precise membrane trafficking mechanisms by which ATG proteins facilitate secretory granule exocytosis in Paneth cells or other cell types remain unknown. However, a recent study indicates that lysozyme is localized in large LC3-positive vesicles in Paneth cells from wild-type but not Atg 16 L1 T300A mice.
Thus, in a manner similar to autophagosome-like vesicles involved in unconventional protein secretion, secretory granules may be earmarked for exocytosis by the presence of LC3 on their surface.
A related, but topologically distinct, link between autophagy and secretory lysosome exocytosis was uncovered in another specialized type of secretory cell, the osteoclast. Osteoclasts resorb bone by a mechanism that involves secretory lysosome fusion with bone-apposed plasma membrane composed of ruffled borders, with the discharge of matrix-degrading molecules into the site of osteal degradation. In mice, the ATG protein conjugation machinery and the Rab GTPase, Rab7, are essential for generating an LC3-labeled ruffled border, cathepsin K release and normal bone resorption, thus indicating a role for ATG genes in mediating polarized secretion of lysosomal contents to the extracellular space. In this scenario, the plasma membrane, not the secretory lysosome, is labeled by LC3. Thus, during secretion, the ATG protein conjugation machinery and resulting lipidated LC3 can function either in the formation of normal secretory granules that properly fuse with the plasma membrane or in the creation of a specialized plasma membrane that fuses with secretory lysosomes.
The predicted clinical outcome of defects in ATG gene-dependent osteoclast functions would be osteopetrosis, a disease marked by abnormally dense bone. Consistent with this prediction, mutations in PLEKHM1, a Rab7 effector, and the v-ATPase alpha 3 subunit involved in lysosomal acidification, are each associated with osteopetrosis in patients. In contrast, aging, which is associated with reduced autophagy in most cell types, is accompanied by osteopenia and osteoporosis in mice and humans.
This may reflect the roles of ATG genes in other cell types in the bone that favor bone growth and normal bone density, including protection against endoplasmic reticulum (ER) and oxidative stress in osteoblasts and osteocytes and maintenance of the proper function of bone mesenchymal stem cells. Thus, studies in bone represent an elegant example of how the autophagic machinery can exert different specialized functions in distinct cell types within a given organ, functions that may have opposite effects, such as bone resorption and bone formation, to orchestrate overall tissue homeostasis. As osteopenia, osteoporosis and associated skeletal fractures are a major cause of morbidity and mortality in aging humans, this area warrants further investigation as a potential clinical target for autophagy upregulation.
Numerous other defects in protein secretion in ATG gene knockout mice have been described, although it is unclear whether they reflect a direct role for ATG genes in autophagy-independent trafficking or more indirect consequences of autophagy deficiency on secretory processes. These include defects in the assembly and secretion of octogonial core proteins which leads to abnormalities in vestibular development and defects in pancreatic beta cell insulin granule morphology and secretion, melanogenesis and pigmentation, and mucus secretion of airway epithelial cells and intestinal goblet cells.
Accumulating evidence suggests that ATG proteins also have pleiotropic effects on the cellular release of exosomes, a process that is mediated by fusion of the multivesicular body (MVB) with the plasma membrane.
Autophagy induction can prevent, whereas ATG gene silencing or pharmacological inhibition can increase, extracellular release of exosomes, including those containing pathogenic protein cargoes, such as alpha-synuclein, prions and amyloid precursor protein. This regulatory mechanism is presumed to involve MVB fusion with auto phagosomes, thereby diverting MVB transport away from the plasma membrane. Increased exosome release in the setting of impaired autophagy may function as an alternative quality control pathway to maintain cellular homeostasis and prevent cell death due to proteotoxicity. However, there are also examples in which ATG genes stimulate exosome production. Atg5, but not Atg7, has been shown to decrease late endosome acidification by disrupting the v-ATPase, thereby promoting the production of exosomes that enhance tumor metastasis. Similarly, the ATG3-ATG12 conjugate which is required for LC3 lipidation during basal, but not starvation, conditions interacts with the ESCRT protein, Alix, and positively controls Alix-dependent exosome biogenesis. Given the expanding repertoire of exosome-dependent processes, including neurodegeneration, immune signaling, metabolism, tumor metastasis and viral infection, the effects of the autophagic machinery on the fate of the MVB lysosomal degradation or exocytosis, may partly underlie the pathophysiological effects of ATG gene mutation.
The ATG machinery modulates retromer function to control the endosome-to-cell-surface recycling pathway. During metabolic stress, LC3 on autophagic structures binds to the RabGAP protein TBC1D5 to sequester it away from an inhibitory interaction with the retromer complex. This sequestration allows retromers to associate with endosomal membranes and mediate plasma membrane translocation of the glucose transporter, GLUT1, a facilitator of glucose uptake. GLUT1 is required for the low levels of basal glucose uptake required to sustain cellular respiration, and its plasma membrane localization normally increases when cells are exposed to low glucose. Perturbation in ATG protein conjugation may significantly cripple this metabolic homeostatic mechanism involving GLUT1 trafficking and, in addition, affect the cell surface localization of other as-of-yet-unidentified receptors.
Autophagy Genes in Other Dynamic Membrane Events.
Non-autophagic functions of ATG genes in membrane trafficking modulate the infection of host cells by viruses, bacteria and other pathogens. These include processes described above such as LAP, which may be partially antagonized by virulent micro-organisms that enter professional phagocytes, and LC3-regulated exocytosis, which is involved in the egress of viruses that either reside inside autophagosomes or whose envelopes become decorated with LC3. Many additional ATG gene-dependent membrane reorganization events, or interference with such events, also regulate infection.
For example, several ATG genes are required for the formation of intracytoplasmic membrane-associated replication factories of certain medically important RNA viruses, such as hepatitis C viru. Similarly, the formation of multi-membranous vacuoles that support replication of the bacterium, B. abortus, involves ATG genes required for class 3 PI3K activity but not those required for LC3 conjugation.
In contrast, IFN gamma inhibits T gondii replication by a process involving LC3, Gabarap lipidation and recruitment of IFN gamma inducible GTPases to the parasitophorous vacuole, where they disrupt the membrane and destroy the parasite’s replicative niche.
Similarly, IFN gamma mediated control of murine norovirus, a model for human epidemics of gastroenteritis, involves labeling membrane-associated viral replication complexes with lipidated LC3 and recruitment of IFN gamma inducible GTPases. Thus, marking replication-associated membrane structures by LC3 conjugation may represent a conserved mechanism underlying IFN gamma mediated control of intracellular pathogen replication. Further understanding of the precise processes by which different subsets of ATG proteins provide or destroy host membranes necessary for different stages of pathogen replication may lead to the development of new anti-infective strategies.
Beyond Membrane Trafficking: Autophagy Proteins Have Other Functions.
The autophagy proteins not only help orchestrate the cross-talk of diverse vesicular trafficking pathways, but also interface with multiple other cellular pathways, including, but not limited to, cell death pathways, cell cycle regulation, and innate immune signaling. The interaction of FIP200 with Atg13 is essential for autophagy in vivo and neonatal survival in mice, but the non-autophagic function is sufficient to maintain embryogenesis through a mechanism involving protection against TNF alpha induced apoptosis. Atg7, independently of its E1-like enzymatic activity and function in autophagy, regulates p53-dependent cell cycle arrest and apoptosis, and the neonatal lethality of Atg7 knockout mice is partially rescued by inhibition of the DNA damage response through deletion of the protein kinase Chk2. In mice, deletion of Atg9a, but not Atg5, results in a defect in necrosis at the bone surface during developmental morphogenesis. The precise mechanisms underlying these, and additional, functions of individual ATG proteins in cell death and cell cycle regulation are not well understood.
ATG proteins regulate inflammatory and immune signaling both through autophagy-dependent mechanisms, such as by the autophagic removal of damaged mitochondria that produce ROS and activate RIG One signaling and the NLRP3 inflammasome, and autophagy-independent mechanisms that generally involve ATG protein interactions with immune signaling molecules. For example, the ATG5-ATG12 conjugate inhibits type One IFN signaling in response to viral infection by binding to the CARD’s, caspase activation and recruitment domains, of RNA recognition molecules such as RIG one and MAV’s.
Similarly, the cytosolic DNA sensing innate immunity pathway mediated by cGAS, cyclic GMP-AMP, cGAMP, synthase and STING, Stimulator of interferon genes, is regulated by autophagy proteins. The generation of cGAMP by cGAS activates ULK1, which phosphorylates and inhibits STING-dependent cytokine production. As unrestrained STING signaling, either via inherited mutations in the ADAR and ribonuclease H2 complex or gain-of-function mutations in STING, causes human auto inflammatory diseases, ULK1 activating agents have been proposed as potential treatments for such disorders. Beclin 1 binds cGAS to suppress cGAMP synthesis and halt interferon production. Atg9a may also function as a negative regulator of innate immune signaling by decreasing the assembly of STING and TBK1 in the presence of double-stranded DNA. Of note, these same RNA-sensing and cytosolic DNA-sensing signaling pathways are activators of autophagy, which is itself an important innate immune effector pathway.
Thus, ATG proteins play a crucial role in both mediating innate immunity and in providing feedback inhibition to fine-tune inflammatory signaling so as to avoid deleterious consequences.
The Selectivity of Autophagy: a Guardian of Cellular Homeostasis.
For nearly half a century, the process of macro autophagy was believed to lack cargo specificity. In fact, the morphological identification of an auto phagosome required visualizing the simultaneous presence of diverse cytoplasmic contents, such as ER, ribosomes and mitochondria inside a double-membraned vacuole. However, a transformative body of work over the past decade has fully dispelled this notion. We now know that there can be extreme specificity in governing the choice of cargo that is degraded by the autophagosome and an intricate system for earmarking and capturing such cargo. This process, termed selective autophagy, may be more crucial in protection against most mammalian diseases than “bulk autophagy”, which is primarily a homeostatic mechanism during nutrient stress.
Many parts of the cell can be “selected” for degradation by autophagy, Figure 2 and Table 2. Numerous studies have reported the selective autophagy of various organelles, including mitochondria, ER, peroxisomes, lipid droplets, ribosomes, midbody rings and the nucleus. Autophagy selectively degrades aggregation-prone misfolded proteins such as those involved in the pathogenesis of certain neurodegenerative, skeletal and cardiac muscle, and liver diseases. In addition, it degrades the individual proteins that serve as adaptors to bridge cargo with the nascent phagophore as well as specific inflammatory and immune signaling molecules. Moreover, selective autophagy can target pathogens that reside inside vacuoles or directly inside the cytosol for lysosomal degradation. Once captured, cargo degradation proceeds through a route that involves the same molecular machinery as bulk autophagy. Different forms of selective autophagy are often named by a term comprising a prefix derived from the cargo. For example, mito, ER, ribo, nucleo, pexo, lipo, and the suffix “phagy”. For selective autophagy of microbial invaders, the term xenophagy is commonly used.
Major advances have been made in understanding certain aspects of selective autophagy, particularly how cargo binds to the forming phagophore, Figure 2.
In most known instances, the cargo either contains an identifiable LC3-interacting region or LIR motif that directly binds LC3, or it must be labeled with a tag such as ubiquitin, which then binds adaptor proteins that contain both a ubiquitin-binding domain and a LIR motif, thus serving as a bridge between the cargo and the LC3, or Gabarap’s, conjugated to the phagophore membrane. Alternatively, specific proteins (particularly those involved in the inflammasome or IFN signaling) can bind to TRIM (tripartite motif) family members, which serve as adaptors that interact with Gabarap’s to target such proteins for autophagic degradation. Of note, the proteins we refer to as “adaptors” are often called autophagy “receptors”. However, as these are bridging molecules that are not integral parts of cellular membranes that undergo ligand-dependent activation, the designation as “receptors” can be confusing.
Several layers of control are needed to properly dictate the targeting of cargo for autophagy. In theory, cargo should be disposed of when it is constitutively harmful, for example, intracellular pathogens, potentially dangerous to the cell, for example, mitochondrial damage, or obsolete as a result of cellular differentiation, for example, organelles during erythrocyte maturation. In the scenario where LC3 directly binds to a protein on an organelle containing a LIR motif, there must exist ways to hide or expose the LIR motif in a regulated-fashion.
Two mechanisms identified thus far include:
(1) Stimulatory and inhibitory phosphorylations of residues near or in the LIR motif (such as occurs for the mitochondrial outer membrane protein, FUNDC1, that mediates hypoxia-stimulated mitophagy and (2) The exposure of a normally hidden LIR motif, such as occurs when proteasomal-dependent rupture of the outer mitochondrial membrane exposes the inner mitochondrial membrane LC3-binding protein, prohibitin 2.
Under circumstances where LC3 binds to an adaptor protein, there must exist ways to recruit the adaptor to the cargo destined for degradation. This process generally involves the concerted action of E3 ligases that ubiquitinate targets, for example, Parkin, SMURF1, kinases that recruit E3 ligases, for example, PINK1, or that phosphorylate LIR domains of adaptors, for example, TBK1, deubiquitinating enzymes, DUB’s that counter E3 ligase activity, for example, USP30, USP15, and acetylation, deacetylation of mitochondrial and ER target proteins.
All mechanisms for earmarking cargo must be tightly coordinated with the formation of autophagosomes to ensure final cargo disposal. Some potential nodes of coordination have recently been described. Certain autophagy adaptors, most notably the TRIM family proteins, bind not only substrate proteins and LC3, Gabarap family members but also assemble the ULK1 and Class 3 PI3K complexes to initiate autophagosome formation. ULK1, a “master kinase” that phosphorylates multiple sites on downstream core autophagy proteins, is recruited to and phosphorylates proteins involved in selective autophagy, such as the LIR domain of the mitochondrial membrane protein, FUNDC1. An organelle-specific LC3, Gabarap binding protein, the ER membrane protein, CCPG1, interacts with a key component of the autophagy-initiating ULK1 complex, FIP200. Thus, the machinery involved in selective autophagy substrate recognition may play an active role in autophagy initiation.
For selective autophagy targeting events that involve substrate ubiquitination, the precise mechanisms that dictate the choice between autophagic versus proteasomal degradation are uncertain. In yeast, substrate aggregation and oligmerization of the ubiquitin-binding proteins may favor autophagic degradation. The lysine residues used for linkage and the length and nature of the ubiquitin chains have also been proposed to contribute to pathway selection, but definitive evidence is lacking. Moreover, despite elegant studies characterizing the ubiquitylome of selective autophagy cargo, such as mitochondria and intracellular pathogens, the ubiquitin substrates required for autophagic targeting remain largely undefined.
Selective autophagy seems to involve multiple concurrent targeting mechanisms that act in a cooperative, potentially hierarchical and, or partially redundant manner to ensure proper removal of cargo. This “combinatorial design” may allow specific cell types to more precisely regulate when and how selective autophagy occurs for a given cargo.
The partial redundancy also renders it more feasible to study loss-of-function phenotypes of genes required for selective autophagy but dispensable for bulk autophagy, as compared to those required for bulk autophagy, as they are less likely to be lethal to the cell or organism. As selective autophagy genes are partially redundant, this may explain why their loss-of-function mutation seems to be better tolerated in the human population than loss-of-function mutation of core ATG genes.
Indeed, mutations in many of the known molecules involved in selective autophagy are associated with susceptibility to a variety of human diseases, Table 1. Mutations in the genes encoding the adaptor proteins p62, SQSTM and optineurin, the E3 ligase Parkin, and the kinases PINK1 and TBK1, are among the most common causes of familial and early onset neurodegenerative diseases, including Parkinson’s disease, frontotemporal dementia and amyotrophic lateral sclerosis. Hereditary sensory and autonomic neuropathy type II is caused by mutations in an ER-specific LC3 binding protein, FAM134B, required for ER-phagy. Mutations in TRIM20, also known as pyrin, that impair its ability to target inflammasome components for autophagic degradation result in an inherited autoinflammatory disorder, familial Mediterranean fever. Inflammatory bowel disease-associated genes encode proteins that function in multiple steps of autophagy, including the selective targeting of bacteria by the adaptor, Calcoco2, NDP52, and the E3 ligase, SMURF1.
The numerous links between mutations in selective autophagy genes and human diseases underscore the likely physiological importance of different forms of selective autophagy, Table 2. However, the precise mechanisms that connect genotype to phenotype remain largely undefined. For example, it is not known why mutations in Parkin and PINK1 are associated with Parkinson’s disease, whereas mutations in optineurin, TBK1, and p62, SQSTM1 are associated with amyotrophic lateral sclerosis and frontotemporal dementia, Table 1.
In addition to potential cell non-autonomous effects of mutations in these genes in tissues outside of the brain, cell type-specific differences in various populations of neurons and glia may exist with respect to:
(1) Dependency on subsets of selective autophagy genes.
(2) Expression and activity of DUBs and other negative feedback mechanisms that regulate selective autophagy, and, or
(3) levels and types of stress that mandate different types of selective autophagy responses to maintain homeostasis, such as mitophagy or other forms of selective autophagy such as aggrephagy that are relevant to neurodegenerative diseases.
Parkin knockout mice, unlike flies lacking Parkin, do not develop spontaneous neurodegeneration, but they do develop dopaminergic neuronal degeneration, resembling that observed in human Parkinson’s disease, when crossed with “mutator” mice with a proof-reading-defective mitochondrial DNA polymerase (PolG) that accumulate mitochondrial mutations. The localization of disease in dopaminergic neurons may be related to increased mitochondrial stress in these cells as compared to other neuronal populations in the brain. Intriguingly, the motor defect and neurodegeneration in Parkin-null, mutator mice can be rescued by deletion of STING, a regulator of Type One IFN responses to cytosolic DNA. Thus, aberrant inflammatory signaling as a result of defects in mitophagy may contribute to the pathogenesis of neurodegenerative disease in patients with Parkin or PINK1 mutations.
While most, if not all, forms of selective autophagy are likely to contribute to normal physiology and protection against disease, mitophagy has been the most extensively studied. Mitophagy is an essential component of mammalian developmental and differentiation processes, including elimination of paternal mitochondria from the fertilized egg, removal of mitochondria during red blood cell maturation and beige-to-white adipocyte differentiation. In addition to Parkinson’s and other neurodegenerative diseases, defective mitophagy is thought to contribute to organ-specific and systemic inflammatory diseases, cancer development and, or progression, and potentially aging. The removal of damaged mitochondria by mitophagy maintains normal cellular metabolism, reduces mitochondrial generation of ROS that trigger inflammation and genotoxic stress, and prevents mitochondrial release of pro-apoptotic factors. Thus, maintenance of proper mitochondrial function by mitophagy is crucial for cellular and organismal health. Other forms of selective autophagy, including xenophagy, likely operate in an analogous manner to mitophagy, in that the mechanisms by which they regulate physiology and disease are a function of the normal “duties” of their substrate and the ensuing pathological consequences of abnormal substrate accumulation, see Table 2.
Our expanding knowledge of the mechanisms and physiological functions of selective autophagy may open up new, albeit unchartered, pathways for drug discovery. Knockdown of the mitochondrial deubiquitinase, USP30, rescues mitophagy defects and disease in flies with pathogenic mutations in Parkin, suggesting a potential role for the inhibition of DUBs that target selective autophagy E3 ligases in the treatment of Parkinson’s and other diseases. Indeed, novel highly selective inhibitors of USP30 that accelerate mitophagy have recently been reported.
As phosphorylation of substrates is also a common mechanism involved in selective autophagic targeting, it may be possible to activate specific kinases to enhance selective autophagy. Potentially, it may also be possible to develop novel strategies to attach high-affinity LIR domains selectively to harmful cargo so that they can be more efficiently captured by an LC3-decorated nascent autophagosome.
Autophagy Regulation: A Nexus for Therapeutics?
Autophagy was originally studied in yeast and mammalian cells as a nutrient stress response pathway. During the past decade, we have dramatically expanded our knowledge of autophagy regulation, particularly the spectrum of physiological and pathophysiological stimuli that control autophagy, the mechanisms that regulate the activity of the core autophagy proteins, and the interconnectivity of autophagy with other cellular stress response pathways. These concepts have been reviewed elsewhere, here, we highlight selected aspects relevant to physiology and disease.
Post-translational protein modifications such as phosphorylation, ubiquitination, and acetylation play a central role in coordinating the activity of ATG proteins. In most cases, the upstream kinases, phosphatases, ubiquitin ligases, DUB’s, and acetyltransferases simultaneously modify both ATG proteins and proteins involved in other cellular stress-response pathways that are co-regulated with autophagy. As a result, pharmacological targeting of these enzymes will elicit broad-based modulation of multiple intertwined stress-response pathways.
Depending on the enzyme and its substrates, such nonspecific targeting may be harmful in some instances, and useful in others.
One important example is the stimulation of AMPK, a low energy-sensing kinase activated by ATP depletion, which phosphorylates multiple proteins to both stimulate catabolic pathways, including autophagy, and restrain anabolic pathways, including mTORC1 signaling, thereby ensuring limitation of ATP consumption and generation of new ATP via breakdown of metabolic products. In recent years, AMPK has been shown to not only activate autophagy through inhibition of mTORC1, but also directly phosphorylate several ATG proteins, including ULK1, ATG9A, Beclin 1, and VPS34. In addition, AMPK promotes mitophagy through effects on ULK1 and stimulates TFEB-dependent activation of the CLEAR, Coordinated Lysosomal Expression and Regulation, network of genes required for autophagy. This pro-autophagic activity of AMPK occurs concurrently with its effects on mitochondrial homeostasis and on lipid and glucose metabolism.
AMPK activation may underlie the beneficial effects of metformin, a drug widely prescribed for the treatment of diabetes. Metformin activates AMPK indirectly through mitochondrial depletion of ATP, and direct AMPK activators that yield effects similar to metformin are in pre-clinical development. The extent to which autophagy stimulation contributes to beneficial effects of AMPK activation in mice or patients is not known, but it seems likely that autophagy represents a critical part of an AMPK-activated hub that protects against various metabolic diseases, including diabetes, obesity, and non-alcoholic fatty liver disorders, as well as certain cancers and aging-related phenotypes. In Drosophila, deficiency of the Beclin 1 orthologue (ATG6) impairs the ability of metformin to prevent intestinal stem cell aging, and lifespan extension by neuronal AMPK expression requires the fly ULK1 orthologue, ATG1. In mice, AMPK upregulation of autophagy is correlated with improved function of aging muscle stem cells, additionally, muscle-specific AMPK deficiency results in defective autophagy, fasting-induced hypoglycemia, and aging-associated myopathy. In yeast, core ATG genes are required for AMPK-mediated lipid droplet degradation and survival during acute glucose deprivation.
The lysine acetylation, deacetylation of ATG proteins has emerged as a central node of autophagic control regulated by metabolic sensors involved in lipid, glucose and protein metabolism. Moreover, this control center may function independently from, but intertwined with, AMPK and mTORC1. During acute nutrient depletion, cells undergo a rapid decrease in levels of cytosolic acetyl coenzyme A (AcCoA), which leads to the deacetylation of cellular proteins. Sirtuin 1, which is downstream of AMPK, deacetylates multiple ATGs, for example, ATG5, ATG7, ATG12, Beclin 1, VPS34, LC3) and thereby promotes autophagy, as does reduced activity of the acetyl transferase EP300. Hence, endogenous activators of sirtuin-1, for example, nicotine adenine dinucleotide NAD plus, endogenous inhibitors of EP300, for example, spermidine, a dietary polyamine), and reduced availability of AcCoA (a rate-limiting step for EP300 function) all stimulate autophagy.
Compounds that act on these pathways, thereby mimicking the effects of caloric restriction, so-called “caloric restriction mimetics”, are an active area of investigation, and genetic evidence suggests that autophagy is essential for their beneficial effects in vivo. Reservatrol, an indirect sirtuin activator, requires the autophagy machinery for its favorable effects on longevity in nematodes. Spermidine-induced autophagy is required for several of its beneficial health effects in model organisms, including lifespan extension in flies, worms, and mice, prevention of cardiac aging in mice, improvement in neuronal function in aging flies, and preservation of myocyte stemness in mice. Moreover, caloric restriction mimetics improve anti-tumor immune surveillance and enhance chemotherapy responses in autophagy-competent, but not autophagy-incompetent, mouse tumor allografts.
Over the past decade, the lysosome, an organelle traditionally viewed as the downstream “workhorse” for autophagosomal cargo degradation, has been shown to also play a crucial role in the upstream regulation of autophagy. The nutrient-sensing kinase complex, mTORC1, detects both cytosolic and intra-lysosomal amino acids through distinct mechanisms to inhibit autophagy. Amino acids, such as arginine, inside the lysosomal lumen are sensed by the amino acid transporter SLC38A9, which interacts with the lysosomal V ATPase, Rag, Ragulator complex to activate mTORC1. This both restrains autophagy during baseline conditions and provides feedback inhibition to terminate autophagic responses to acute nutrient depletion. mTORC1 activation in the fed state and, or hyperactivation, as a result of mutations in regulatory signals, switches the cell to a state of anabolic growth and energy storage. Although essential for cell growth and proper metabolic regulation, sustained mTORC1 activation at the organismal level is associated with a variety of pathophysiological consequences, including impaired neonatal gluceoneogenesis and survival, accelerated age-related decline in pancreatic beta-cell function, late-onset muscle atrophy, altered lipogenesis and adipogenesis, immune suppression, epileptic seizures and autistic traits, tumorigenesis, and aging.
While in some cases, impaired induction of autophagy has been documented in mice with hyperactive mTORC1 signaling and is postulated to contribute to pathological phenotypes, for example, impaired neonatal gluconeogenesis, late-onset muscle atrophy, the precise role of autophagy inhibition in most diseases associated with mTORC1 signaling remains unknown. There has been some interest in using FDA-approved mTOR inhibitors for the treatment of neurodegenerative disorders that may benefit from autophagy induction. However, the safety and efficacy of using mTOR inhibitors to induce therapeutic autophagy is uncertain, given the broad range of essential catabolic functions regulated by mTORC1 along with the lack of full specificity of existing agents to target mTORC1 rather than mTORC2, which functions primarily as an effector of insulin, PI3K signaling.
Both AMPK and mTORC1 participate, in opposite directions, in a signaling axis that links autophagy, the lysosome, and the transcription factor EB (TFEB) and related family members. During the acute response to autophagic stimuli, transcriptional activation is not required, as evidenced by the observation that enucleated cells (cytoplasts) undergo autophagy.
However, sustained autophagy requires TFEB, a transcription factor that (when inactive) binds to Ragulator at the lysosomal membrane, is phosphorylated by mTORC1, and retained in the cytoplasm by 14 3 3 proteins.
Following mTORC1 inhibition, TFEB dephosphorylation releases it from the cytoplasm, allowing its nuclear translocation and subsequent activation of the CLEAR gene network, which includes genes encoding lysosomal hydrolases, lysosomal v-ATPase pumps, lysosomal regulators and autophagy regulators. As noted above, AMPK also activates TFEB-dependent gene expression, this occurs through multiple different mechanisms. In addition, a recent study showed that phosphorylation of acetyl-CoA synthetase 2 (ACSS2) promotes its transport into the nucleus, where it binds to TFEB and favors the acetylation of histone H3 residues within the promoters of TFEB target genes.
In addition to TFEB, other transcription factors from the same family, such as micropthalmia-associated transcription factor, MITF, and TFE3, or from other families, for example, FOXO3A, HSF1 or TP53, stimulate autophagy. Bromodomain 4, BRD4, a transcription factor that represses autophagy and lysosomal genes, is displaced from chromatin in response to starvation by a signaling cascade involving an AMPK-SIRT1 axis. Thus, multiple known, and probably yet-to-be-identified, transcription factors regulate the synthesis of genes required for autophagy, including both the formation of the autophagosome and degradation of its contents by lysosomes. Not surprisingly, the activity of these transcription factors is tightly regulated by numerous signaling factors that also regulate core ATG protein function by post-translational modifications.
Modulation of the activity of TFEB, a master regulator of both lysosomal biogenesis and autophagy, has emerged as a potential therapeutic strategy. Conceptually, this approach is attractive, since limitations in lysosomal numbers and function either occur intrinsically as part of many rare, but devastating, difficult-to-treat, primary diseases, such as lysosomal storage disorders, LSD’s, or are acquired during the progression of diseases associated with the clearance of toxic aggregates progress, such as Huntington’s, Parkinson’s, Alzheimer’s disease and tauopathies. In mice, TFEB overexpression ameliorates several LSDs, neurodegenerative diseases, and alpha 1-antitrypsin deficiency, and it also promotes lipophagy, thereby reducing obesity and associated metabolic syndrome. The mechanisms by which TFEB overexpression partially corrects lysosomal malfunction in LSDs are not fully understood, but may involve induction of lysosomal exocytosis for the secretion of undigested material.
One potential obstacle to strategies for enhancing TFEB family activity is the risk of tumorigenesis associated with constitutive activation, for example, renal clear cell carcinoma with TFEB and pancreatic cancer with MITF, TFE3, and TFEB. While MITF, TFE3, TFEB-dependent autophagy-lysosomal activation is thought to sustain metabolic reprogramming in pancreatic cancer cells by maintaining intracellular amino acid pools, further genetic investigations are warranted to confirm that ATG genes are involved in these effects. Moreover, enhanced activity of BRD4, a transcriptional repressor of autophagy, drives another type of cancer, NUT midline carcinoma, suggesting the effects of transcriptional regulators of autophagy on tumorigenesis may be cell-type specific.
It is unclear whether specific subsets of the TFEB-regulated gene network can be induced to avoid genes that contribute to tumorigenesis without losing beneficial effects on the autophagy-lysosomal pathway. An alternative strategy is to activate TFEB on an intermittent basis and, or for limited periods to avoid potential oncogenic effects.
Intriguingly, one of the most widely used medications in the world, aspirin, has been reported to upregulate TFEB in brain cells, via activation of PPARC alpha, induce lysosomal biogenesis, and decrease amyloid plaque pathology in a mouse model of Alzheimer’s-like disease. Aspirin (and its active metabolite salicylate) also induces autophagy via inhibition of the acetyltransferase EP300 and via AMPK activation, mTORC1 inactivation. However, there is as-of-yet no direct genetic evidence that autophagy contributes to the health benefits of aspirin.
Highly specific activation of autophagy may be possible through strategies that enhance the activity of the upstream components in the core autophagy pathway, meaning the ULK1 serine, threonine kinase complex and, or Beclin 1, VPS34 lipid kinase complexes. As a key allosteric regulator of VPS34 lipid kinase activity, Beclin 1 activity is tightly regulated by multiple post-translational modifications, ubiquitination, acetylation, phosphorylation, which govern its stability, heterodimeric binding to ATG14 or UVRAG, homodimerization in an inactive form, and, or binding to negative regulators.
Diverse stress kinases, including AMPK, as well as the upstream ATG protein, ULK1, mediate stimulatory phosphorylations of Beclin 1. The oncogenic kinases, Akt and EGFR, and the EP300 acetylase inhibit the autophagic activity of Beclin 1, mutation of their target post-translational sites in Beclin 1 demonstrates that suppression of Beclin 1-dependent autophagy promotes tumor growth in mouse xenograft models.
Enhanced proteasome-mediated degradation of Beclin 1 due to decreased binding of the deubiquitinase, ataxin 3, may contribute to dysregulated autophagy in cells of patients with polyglutamine expansion protein-related diseases, such as Huntington’s and spinocerebellar ataxia type 3..
Disruption of Bcl-2 binding to Beclin 1 represents a central mechanism by which autophagy is activated in response to stress stimuli, such as starvation, exercise, and immune signaling. This disruption can be triggered by phosphorylation of the BH3 domain of Beclin 1 by DAPK, ubiquitination of the Beclin 1 BH3 domain by the E3 ligase TRAF6, phosphorylation of Bcl-2 by JNK1, or competition by BH3-only proteins. Mice containing knock-in non-phosphorylatable mutations in Bcl-2 that prevent disruption of its binding to Beclin 1 are deficient in starvation and exercise-induced autophagy, have decreased exercise endurance, and fail to manifest the beneficial effects of exercise on glucose metabolism.
Conversely, mice with a knock-in mutation in Beclin 1 that decreases Bcl-2 binding exhibit increased autophagy and extended lifespan and healthspan, including protection against Alzheimer’s-like disease and HER2-mediated breast cancer. Thus, disruption of Beclin 1, Bcl-2 binding may be a safe and effective approach to induce autophagy in vivo, preclinical studies are in progress to develop agents that act through this mechanism.
Cell-penetrating peptides, Tat-Beclin 1, derived from a flexible hinge region of Beclin 1 important for VPS34 membrane association and lipid kinase activity are sufficient to induce autophagy in vitro and in vivo. In mice, Tat-Beclin 1 protects against West Nile virus, chikungunya virus and E. coli bacterial infections, lipopolysaccharide-induced cardiac dysfunction, pressure overload-induced heart failure, hyperammonemia in liver failure and urea cycle disorders, and bone loss in LSDs and in FGF-deficiency.
It also enhances chemotherapeutic effects of murine cancers in immune competent mice, reduces the growth of human HER2-positive breast cancer xenografts in immune-deficient mice, and acts synergistically with erastin to increase animal survival in an orthotopic pancreatic cancer model. In rats, intrahippocampal injection of Tat-Beclin 1 improves long-term spatial memory. In a zebrafish model of human polycystic kidney disease, Tat-Beclin 1 ameliorates renal cyst formation. Further studies are needed to examine whether Tat-Beclin 1 induces these effects through autophagy, autophagy-independent effects of Beclin 1, or alternative mechanisms. Moreover, precise definition of its mechanism of action may lead to the development of novel small drug-like molecules that mimic its activity. Recent structural advances elucidating the atomic details of the Beclin 1, VPS34 complexes may provide a basis for rational drug design to selectively activate autophagy-specific Beclin 1-associated VP34 lipid kinase activity.
Autophagy in Tissue and Whole-Body Homeostasis.
The health of multicellular organisms requires the coordinated regulation of cellular life and death decisions, cell fate determinations, preservation of genomic integrity, immune responses, and metabolic circuitries. The autophagy machinery, via its diverse functions described above, and yet-to-be-discovered mechanisms, plays a crucial role in these processes. Herein, we highlight some recent advances related to the role of autophagy in cell death, preservation of stem cells, tumor suppression, longevity and defense against metabolic diseases.
Autophagy as a Homeostat
During both routine “housekeeping” and responses to acute stress, cells must find ways to maintain adaptive cytoprotective levels of autophagy while simultaneously avoiding potentially maladaptive levels and, or detrimental effects of autophagy. This balance involves self-control of the levels of autophagy, mechanisms of preventing degradation products from becoming toxic to cells, avoidance of degrading essential cargo, and suppression of unwarranted cell death, which likely is a combined function of the aforementioned processes. Cellular self-titration of levels of autophagy involves multiple different inhibitory feedback loops, including feedback regulation of nutrient sensing signals by the generation of amino acids, acetyl-CoA and respiratory substrates, cytosolic retention of pro-autophagic transcription factors by ATG7, and TFEB-mediated activation of mTORC1. Cellular toxicity by degradation products may be avoided during autophagy, as evidenced by the observation that the generation of lipid droplets generated by autophagy-dependent dismantling of lipid membranes during starvation-induced autophagy sequesters fatty acids, thereby protecting mitochondria against lipotoxicity and preserving cellular viability.
It is not known whether starvation-induced autophagy preferentially induces removal of certain, perhaps aged, structures, and if so, by what mechanisms, or whether it is non-specific. Mitochondria elongate during starvation, which spares them from the autophagic capture that generally occurs after fission. Numerous yet-to-be-discovered mechanisms likely protect mitochondria and other organelles from excessive autophagic capture during stress-induced autophagy.
The aforementioned negative feedback loops restrain autophagy to adaptive (rather than maladaptive) levels, allowing this homeostatic pathway to exert cytoprotective effects during stress, and thereby, prevent apoptotic and necroptotic cell death. In addition, ATG gene-dependent processes, such as increased plasma membrane localization of the GLUT1 glucose transporter, may increase the threshold of damage required to kill cells and thereby promote successful organismal adaptation to stress. Promotion of cell survival in vivo during stress-induced autophagy may depend on concurrent antagonism of the sodium, potassium ion, ATPase pump, which normally consumes a large fraction of the ATP available to the cell. Interestingly, during acute bouts of exercise or nutrient limitation, potent physiological stimuli of autophagy that generally do not result in cell death, endogenous cardiac glycosides that target sodium, potassium ion ATPase are upregulated 50 to 500 fold, resulting in decreased cellular ATP consumption.
However, when organisms are pushed beyond physiological limits of energy deprivation, adaptive mechanisms are insufficient to keep cells alive and to prevent tissue damage. In the liver of patients with anorexia nervosa or in neonatal rodents subjected to severe cerebral ischemic injury, a morphologically and genetically distinct form of cell death occurs called autosis, which requires both ATG genes and sodium, potassium ion, ATPase activity. It is not clear how the cell’s major consumer of ATP, meaning the sodium, potassium ion, ATPase pump) and the cell’s major mobilizer of ATP-generating substrates during stress conditions, meaning autophagy, interact to regulate life and death decisions of the cell. However, this interaction may represent a fundamental energy homeostatic mechanism that becomes pathologic during different types of ischemic conditions.
Another recently identified bona fide form of autophagic cell death, meaning demonstrating a genetic requirement for ATG genes, involves GBA1, the gene encoding the lysosomal enzyme, glucocerebrosidase (GCase, which metabolizes glucosylceramide (GlcCer) to ceramide and glucose. GB
-
2:10:55
FreshandFit
10 hours agoAndrew Branca And Donovan Sharpe COOK Some Dumb Hoes!
182K95 -
22:26
Liberty Hangout
11 hours agoAnti-Trumper Has Complete Meltdown
18.6K46 -
2:21:24
Badlands Media
14 hours agoDevolution Power Hour Ep. 374: Declassified Intel, Trump’s Traps, and the Optics of Collapse
129K38 -
2:00:38
Inverted World Live
8 hours agoWhite House Boosts the AI Revolution | Ep. 79
48K13 -
10:23:56
Dr Disrespect
17 hours ago🔴LIVE - DR DISRESPECT - 10 WINS CHALLENGE - BIG ANNOUNCEMENT AT 12PM PT
162K20 -
1:35:15
Man in America
11 hours ago🚨 ALERT: Hospitals in the U.S. Are KILLING Patients… for Their Organs!
65.8K24 -
2:36:28
I_Came_With_Fire_Podcast
15 hours agoObama's Treason, Trade War: Season Infinity, and Hunter's Pipe Dream
30.7K1 -
2:52:51
TimcastIRL
8 hours agoObama Referred To DOJ For TREASON, Criminal Investigation, CIVIL WAR!! | Timcast IRL
236K144 -
9:43:32
RalliedLIVE
15 hours ago $10.31 earned10 WINS WITH THE SHOTTY BOYS
111K7 -
1:35:43
Badlands Media
19 hours agoAltered State S3 Ep. 38: Biolabs, Bitcoin & the Epstein Data Vault
69.5K15